Humans, like many animal species, can see colors. For primates, this capacity is believed to have been acquired throughout evolution to find food (young leaves, ripe fruits), detect predator camouflage1, or notice social information through peers’ facial coloration2.
How does color vision work? And why do people with color vision deficiency have trouble telling the difference between some colors? Let’s find out!
Color perception starts with the ability to detect light wavelengths
The magic of sight takes place in the retina, a light-sensitive tissue at the back of the eye. That’s where two main types of photoreceptor cells are: rods for vision in low light conditions and cones for sight in bright light conditions. The latter, cone cells, are those responsible for color vision.
There are three sorts of cones, each expressing a different variant of a protein called opsin: S-opsin, M-opsin and L-opsin. These proteins are located in cone cell membranes and bond to a molecule that absorbs light (a chromophore) to form a light-sensitive pigment. Depending on the opsin variant they are formed from, these pigments are sensitive to different wavelengths of light: Short wavelengths for S-pigments, Middle wavelengths for M-pigments and Long wavelengths for L-pigments.
Each type of photopigment can absorb light wavelengths in a certain range, with a peak sensitivity (maximal absorption efficiency) at about 420 nm (S-pigment), 530 nm (M-pigment) and 560 nm (L-pigment).
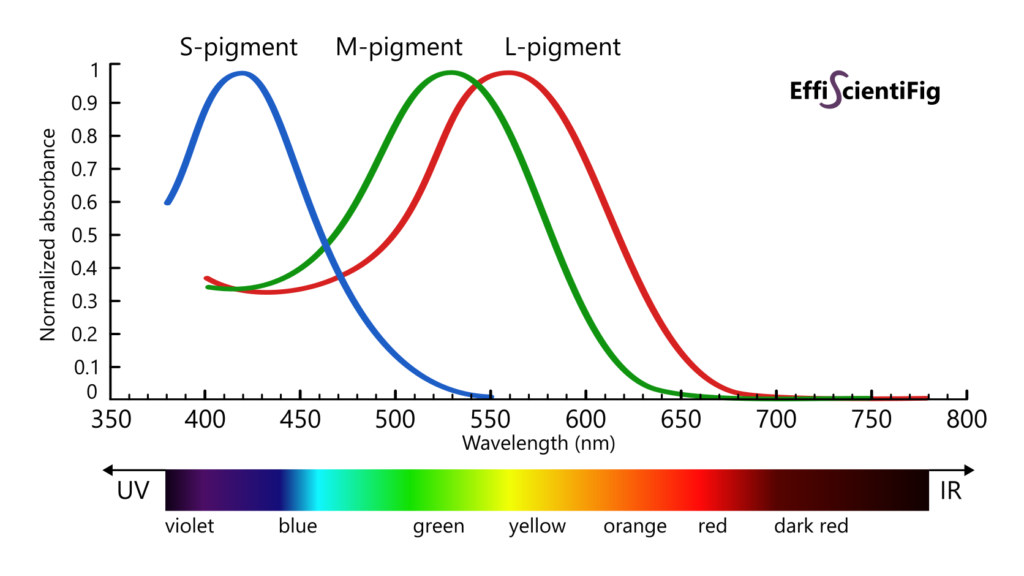
Light absorption by a photopigment initiates a reversible transformation of the chromophore. This structural modification in turn leads to a change in spatial conformation of the bounded opsin, which activates a cascade of signals. The electric impulses generated from cone cells run to ganglion cells, to the optic nerve and eventually reach the occipital lobe at the back of the brain, where the visual information is interpreted. It is the combination of all the signals coming from the cone cells that induces color perception: the efficiency of each cone class to absorb a given wavelength dictates their degree of simulation and thereby determines the color we perceive.
Out of simplification, the cones classes are often designated with colors: blue for S-cones, green for M-cones and red for L-cones. Nonetheless, this naming does not match exactly the color corresponding to their peak sensitivity and masks the considerable overlap between the action spectra of the three cone types.
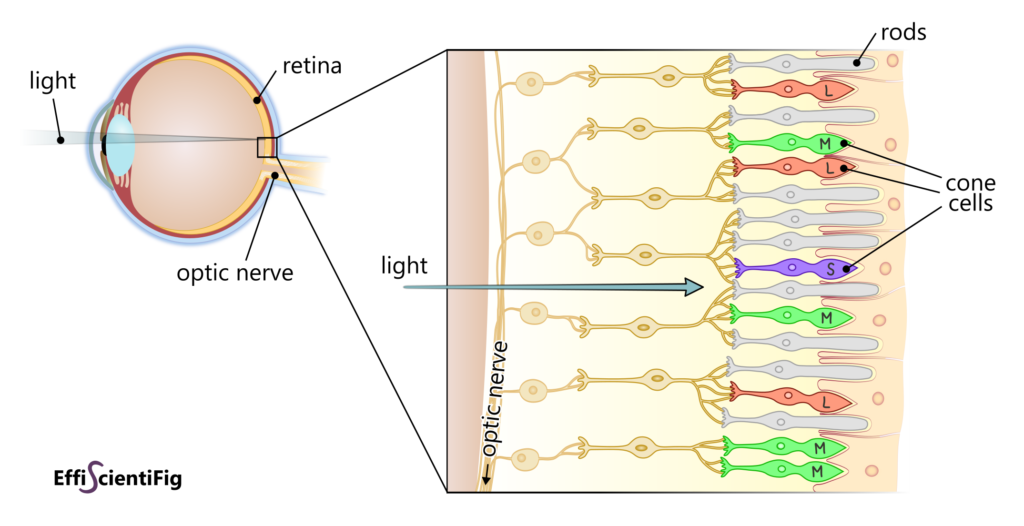
Thanks to these three types of cone cells, human vision is trichromatic, with the ability to detect wavelengths from 380 to 780 nm. Other vertebrates such as birds have a tetrachromatic vision: they present four types of cone cells and can perceive UV wavelengths3. However, most mammals and some color-blind humans are dichromats.
Color vision deficiencies: when some cone cells cannot do their job
There are several types of color vision deficiencies, with different degrees of severity.
The eyes of persons who experience dichromatic vision possess the three types of cone cells, but the gene coding for one of the opsin types is absent from their DNA sequence, or severely altered. Thus, for these individuals, the absence of one of the opsin types prevents the corresponding cone cells from contributing to color vision. The induced color vision defects are called tritanopia (blue blindness), deuteranopia (green blindness) and protanopia (red blindness) when S-opsins, M-opsins or L-opsins are missing, respectively.
Sometimes the color vision defect is only partial and causes anomalous trichromacy. Similarly to dichromatic vision, one of the cone cell types does not contribute to vision (often the M- or L-cones), but one of the other cone type (L or M) produces two versions of L- or M-pigments: a normal pigment and an anomalous pigment that presents a similar, but non-identical spectral sensitivity4.
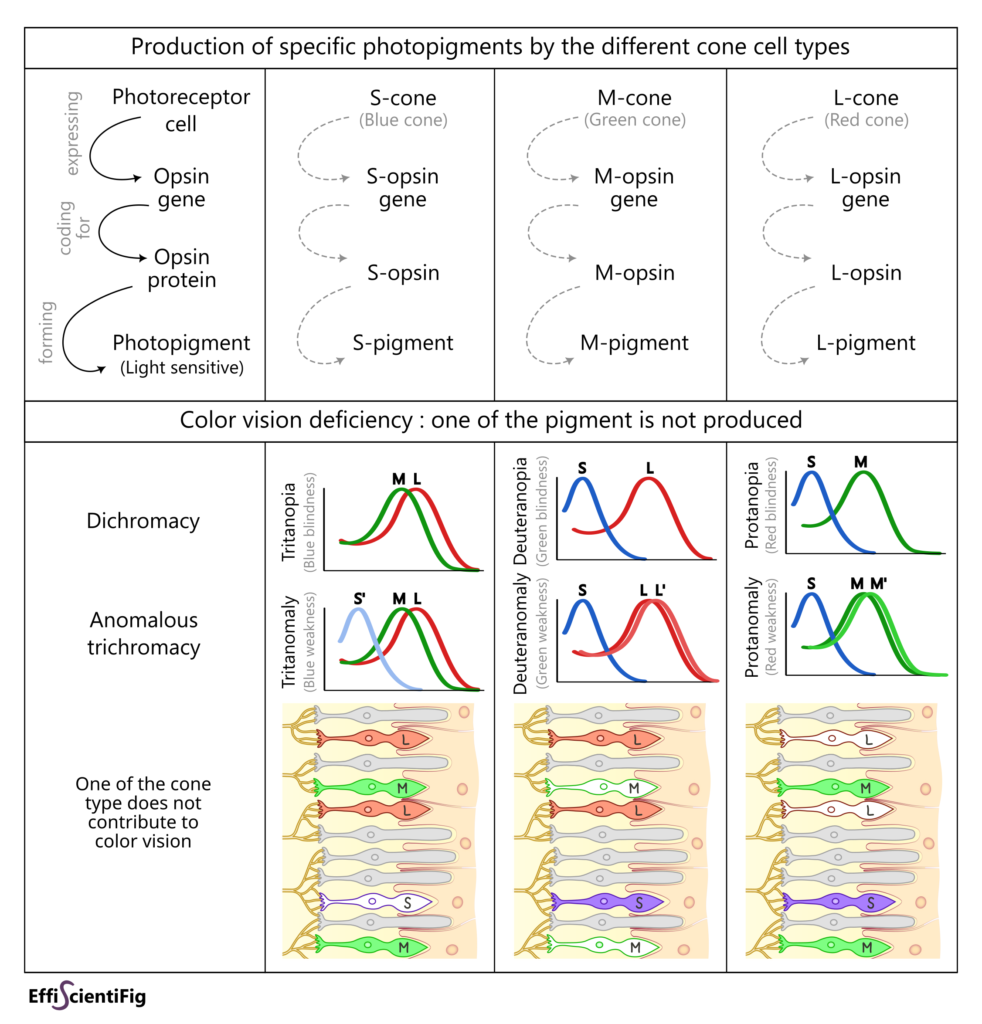
The above examples (dichromacy and anomalous trichromacy) are the most common forms of color vision anomalies. In much rarer cases, some people experience monochromatic vision (only one cone cell type is functional) or even achromatopsia (none of the three cone cell types is functional): these persons see in shades of grey.
A high frequency explained by genetic specificities
Color vision deficiency affects ~5% of people, i.e. about 1 in 20 persons. That represents a whole lot of people. To better picture this number, I like to keep in mind the striking example that in any classroom, there is a great probability at least one person is colorblind. You can test this prevalence yourself by asking around you, you might be surprised to find out that some of your acquaintances or collaborators experience color vision deficiency.
There is a great chance that these persons are men or people assigned male at birth: for the two most common types of color blindness (protan and deutan deficiency, aka red-green color deficiency) the genes coding for L-opsin and M-opsin are carried by chromosome X.
Since XY individuals possess a single copy of the X chromosome, the absence of L- or M-opsin gene (or presence of an altered copy) is not compensated by the presence of a normal copy of the gene on a second X chromosome. Therefore, males are more prone to present a red or green color vision defect, and it concerns about 8% of them.
Red-green blindness is inherited from the mother (or birthing parent) that often does not present color vision deficiency. Indeed, for XX individuals, red-green vision deficiency happens only if the two copies of the X chromosome are impacted by a deleterious opsin gene mutation. In this case, both parents have to carry an impacted X chromosome; the probability of this combination to occur is low, which decreases the prevalence of red-green color blindness to 0.4% in XX individuals.
Interestingly, the L- and M-opsin genes are physically close on chromosome X and their DNA sequences are almost identical (98% similar), which explains the high rate of anomalies possible through recombination events4
The blue-yellow (tritan) color deficiency is rarer (1 in 500 persons5) and affects XX and XY individuals indifferently given that the gene coding for the S-opsin is on chromosome 7 and not on a sexual chromosome.
The overall above-cited prevalence figures seems to vary by ethnicity and are more applicable to people with Northern European ancestry, who tend to present the highest prevalence of color blindness5.
Most of the time, color vision deficiency comes from genetic characteristics inherited from one or both biological parents. Nonetheless, it can also occur due to a disease (cataracts, macular degeneration, alcoholism…).
It’s all about nuances
Overall, color vision varies from one person to another. Indeed, we do not possess the same quantity of each cone type. L-cones are always the most numerous (~64%), followed by M-cones (~33%), and finally S-cones (~3%)6, although these proportions vary greatly from one person to another, especially between L and M cones7. In addition, there is a certain variability in the normal pigments produced by cone cells depending on people, which induces thin differences in their spectral sensitivity4.
This is why the color of an element can be discriminated differently even between two persons experiencing normal color vision. That’s how you get conversations such as:
«I like the purple shirt you are wearing!
– What do you mean? This blue shirt?»
or «I told them to look for the green door in the street.
– How funny, I would have said it’s a turquoise door».
Color perception is relative, it is just an interpretation from our brain!
The above applies to people experiencing color vision deficiency as well. Therefore, two persons can both be affected by deuteranopia and not distinguish colors the same way. Sometimes, differentiating between two colors will be a matter of the amount of light. For anomalous trichromacy, the variance in the anomalous pigments also comes into play since the anomaly can be different from one person to another.
Contrary to the widespread belief, for people experiencing red-green colorblindness, reds and greens are not always completely indistinguishable. However the perceived colors for red and green are often less contrasted than red and green are.
In the example below, the colors on the left are as seen with normal vision, and the two columns on the right are a simulation of these colors as they may be perceived by someone affected by protanopia or deuteranopia. You can see that for two upper colors the simulation results in hues that are in the same tone but that are still distinguishable from one another thanks to their greater brightness. However, the two bottom colors are barely different for a person with protanopia or deuteranopia.
For protanopia and deuteranopia, there is also the combination between yellow and green or between purple and blue that can be problematic:
Making your work accessible to people experiencing color vision deficiency is not just about avoiding using green and red. Thankfully, many tools exist to help choose appropriate colors so you can properly convey the right information to a maximum of persons.
Learn more about useful resources to make your projects more accessible and inclusive,
whether for plots, maps, or any other type of design
References
1 Carvalho, L. S., Pessoa, D. M., Mountford, J. K., Davies, W. I., & Hunt, D. M. (2017). The genetic and evolutionary drives behind primate color vision. Frontiers in ecology and Evolution, 5, 34.
2 Hiramatsu, C., Melin, A. D., Allen, W. L., Dubuc, C., & Higham, J. P. (2017). Experimental evidence that primate trichromacy is well suited for detecting primate social colour signals. Proceedings of the Royal Society B: Biological Sciences, 284(1856), 20162458.
3 Kelber, A. (2019). Bird colour vision–from cones to perception. Current Opinion in Behavioral Sciences, 30, 34-40.
4 Neitz, J., & Neitz, M. (2011). The genetics of normal and defective color vision. Vision research, 51(7), 633-651.
5 Simunovic, M. Colour vision deficiency. Eye 24, 747–755 (2010).
6 Ding, X., Radonjić, A., Cottaris, N. P., Jiang, H., Wandell, B. A., & Brainard, D. H. (2019). Computational-observer analysis of illumination discrimination. Journal of Vision, 19(7), 11-11.
7 Roorda, A., & Williams, D. R. (1999). The arrangement of the three cone classes in the living human eye. Nature, 397(6719), 520-522.